Peking University, June 28, 2023: As a macroscopic quantum state of matter, superconductivity has attracted tremendous attention in the field of scientific research and industry over the past century. According to the BCS (Bardeen-Cooper-Schrieffer) microscopic theory, superconductivity arises from the condensation of coherent Cooper pairs, and each Cooper pair is formed by two electrons with opposite spins and momenta. Theoretically, when time-reversal symmetry is broken, Cooper pairs may acquire a finite momentum and exhibit a spatially modulated superconducting order parameter, which is known as the Fulde-Ferrell-Larkin-Ovchinnikov (FFLO) state. Although the FFLO state was theoretically proposed in 1964, it has proven challenging to observe the FFLO state due to the stringent requirement for materials. To date, direct evidences of the FFLO state, such as the modulation of the superconducting order parameter in real space, have not been detected experimentally.
To understand the observed two-dimensional (2D) superconducting properties in cuprates, some theoretical works predicted that the finite-momentum Cooper pairs can exist in strong-coupling systems without breaking time-reversal symmetry and show the spatial modulation of Cooper-pair density. This extraordinary superconducting state, referred to as the pair density wave (PDW), has sparked numerous theoretical investigations due to the potential connection between the PDW and unconventional superconductivity. Among various theoretical hypotheses, the most intriguing one is that the PDW is another principal state along with d-wave superconductivity in the phase diagram of cuprates, which provides new insights into the complex intertwined orders of the cuprates showing high-temperature superconductivity. Moreover, according to some theoretical proposals the enigmatic pseudogap phase of cuprates can be attributed to the PDW state, further indicating the potential importance of PDW. However, experimental evidences of the PDW state in high-temperature (high-
Tc) superconductors have only been observed in some cuprates. The existence of PDW state in iron-based superconductors, another high-
Tc superconductor family, has never been experimentally detected. Furthermore, early theoretical studies of cuprates proposed the PDW is a low-dimensional stripe order in 2D systems, but no compelling experimental evidences of the PDW in 2D systems have been reported so far.
Recently, Prof. Jian Wang’s group at Peking University, in collaboration with Prof. Ziqiang Wang at Boston College and Prof. Yi Zhang at Shanghai University, discovered the primary pair density wave state in a 2D iron-based high-
Tc superconductor, which provides a new 2D high-
Tc platform to investigate the PDW in unconventional superconductors. By using molecular beam epitaxy (MBE) technique, Jian Wang’s group successfully grew large-area and high-quality one-unit-cell-thick Fe(Te,Se) films on SrTiO3(001) substrates (1-UC Fe(Te,Se)/STO), which show superconducting gap as large as 18 meV, much higher than that (~1.8 meV) in bulk Fe(Te,Se), a promising topological superconductor candidate. Previously, Jian Wang group and collaborators discovered zero-energy excitations at both ends of 1D atomic line defects in 1-UC Fe(Te,Se)/STO, which are found to be consistent with the Majorana zero modes interpretation (Nat. Phys. 16, 536-540 (2020)). In the current work, another atomic structure in 1-UC Fe(Te,Se)/STO, the innate domain wall where the atomic lattice is compressed along Fe-Fe direction across the domain wall (Fig. 1a-d), was investigated by in situ low-temperature (4.3 K) scanning tunneling microscopy/spectroscopy (STM/STS). Within the domain wall area, clear spatial modulation of the local density of stats (LDOS) is detected (Fig. 1e-f). By performing the 2D lock-in analysis (Fig. 1g-h), a modulation period of 3.6
aFe (
aFe is the distance between neighboring Fe atoms) is determined. Further bias voltage dependent measurements show that the period of the LDOS modulation is independent of the energy, demonstrating an origin of electronic order. Furthermore, the electronic ordering-induced LDOS modulations mainly exist in the energies within the superconducting gap, indicating that the charge order is potentially related to the superconductivity of 1-UC Fe(Te,Se)/STO.
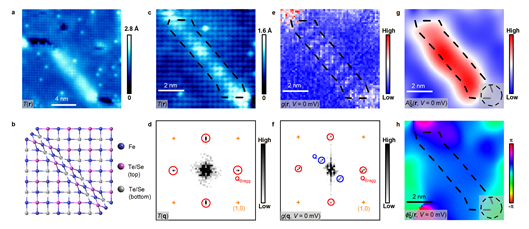
Figure 1 (a) An STM topographic image of the domain wall structure (bright line) in 1-UC Fe(Te,Se)/STO. (b) The schematic of the domain wall in 1-UC Fe(Te,Se)/STO, illustrating the compression across the domain wall. (c) Zoom-in image of a, clearly showing the topography of the domain wall D1. (d) The magnitude of the Fourier transform of c. Bragg peaks of the Te/Se lattice are circled in red. (e) Zero-bias conductance (ZBC) map
g(r,
V = 0 mV) taken at the same area in c at 4.3 K, which shows an emergent electronic modulation along the domain wall. (f) The magnitude of the Fourier transform of e. The modulation wavevector Q circled in blue reveals a spatial modulation of LDOS along the direction of the domain wall. (g) Spatial distribution of the LDOS modulation amplitude calculated by the 2D lock-in method, showing the LDOS modulations at Q characterized by higher amplitude (red region) mainly exist within the domain wall region. (h) Spatial distribution of the LDOS modulation phase, showing uniform phases within the domain wall area.
By performing further STS measurements, spatial modulations of the superconducting coherence peak height (Fig. 2a-c) and gap energy (Fig. 2d-f) are detected at the domain wall. Previous studies have reported the strong correlation between these two physical quantities and superconducting order parameter. Therefore, the spatial modulation of the superconducting order parameter is directly observed in real space, which provides compelling evidence of the existence of PDW order in the 2D iron-based high-temperature superconductor.
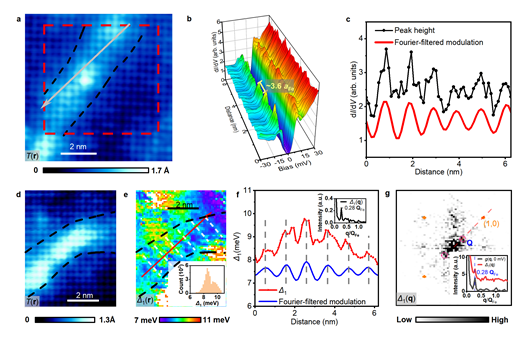
Figure 2 (a) The STM topography of D2. (b) 3D color plot of the d
I/d
V spectra taken along the light grey arrow in a. The periodic modulation of the coherence peak height is detected. (c) Measured height of coherence peak in b (black curve). Red curve is the extracted coherence peak height modulation by applying Fourier filter. (d) STM topography of D3. (e) Spatial distribution of the superconducting gap energy measured in the same area in d. The inset is the histogram of superconducting gap energy. (f) Superconducting gap energy along the red arrow in e (red curve). Blue curve is the extracted gap modulation by applying Fourier filter. Inset: the magnitude of the Fourier transform of the red curve in f, showing a sharp Fourier peak at Q ~ 0.28QFe, corresponding to spatial modulations with a period around 3.6aFe. (g) The magnitude of the Fourier transform of e. Two Fourier peaks at Q ~ ±0.28QFe are marked by red circles. The inset shows the line profiles of Fourier transform of ZBC (red curve) and gap map (black curve) along the (0,0) to (1,0)QFe direction. Peaks at Q ~ 0.28QFe appear for both curves.
Apart from the PDW state, a charge density wave (CDW) state with a period of about 1.8aFe (half of the period of PDW) is also detected at the domain wall. Fig. 3e and 3f show the phase map of the PDW (period ~ 3.6
aFe) and CDW state (period ~ 1.8
aFe) at the domain wall, in which vortices with 2π phase winding in the CDW phase (black dots in Fig. 3e) and π-phase shifts in the PDW phase (arrows in Fig. 3f) can be identified. It is clear that the π-phase shifts in the PDW phase are observed near the vortices of the CDW, which is consistent with the theoretical scenario of a primary PDW and PDW-induced secondary CDW (Fig. 3a-d). Therefore, the PDW state observed at the domain wall of 1-UC Fe(Te,Se)/STO is a primary state.
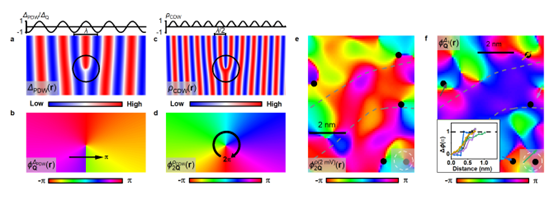
Figure 3 (a) Schematic of a primary PDW order parameter with a half-dislocation. The period of the PDW is λ and the wavevector is
Q. The half-dislocation is circled in black. (b) Spatial phase of
a. The π-phase shift is marked by the black arrow. (c) Schematic image of the secondary CDW induced by the PDW state in
a. The period of CDW is λ/2 and the wavevector is 2
Q. The topological defect (vortex) is circled in black. (d) Spatial phase of
c, showing the 2π phase winding of the 2
Q CDW vortex. (e) Experimental spatial variation of the 2
Q CDW phase in domain wall D3. The vortices are marked by black dots. (f) Experimental spatial variation of the PDW phase at
Q in domain wall D3. The CDW vortices marked by black dots in e are plotted on top of
f. The arrows in
f indicate PDW π-phase shifts, which are in proximity to the CDW vortices, consistent with the theoretical scenario shown in
a-
d. The inset is the evolution of the phase along the arrows in
f.
To explain the mechanism of the primary PDW state at the domain wall, Prof. Ziqiang Wang and Prof. Yi Zhang proposed a novel triplet equal-spin pairing model. At the domain wall, the broken inversion and mirror symmetry introduce the Rashba and Dresselhaus spin-orbit couplings (SOC). Due to the large SOC, electrons with equal spin can pair across the Fermi points of the SOC splitting bands, leading to a primary PDW state with finite-momentum Cooper pairs. Theoretical calculations based on the equal-spin pairing model show the spatial modulation of the LDOS and the superconducting gap, which are consistent with our experimental results and reveal the possible existence of topological spin-triplet superconducting order parameters.
The paper was published online by
Nature on June 28, 2023 (DOI:
10.1038/s41586-023-06072-x). Link to the paper:
https://www.nature.com/articles/s41586-023-06072-x. Prof. Hong-Jun Gao and Prof. Hui Chen at the Institute of Physics of the Chinese Academy of Sciences published a comment entitled
"Widespread pair density waves spark superconductor search" in "News & views" of the same issue of Nature to highlight this work along with three other related works. Prof. Jian Wang at Peking University and Prof. Ziqiang Wang at Boston College are the corresponding authors. Dr. Yanzhao Liu and Tianheng Wei at Peking University contributed equally to this work. Other collaborators include Dr. Guanyang He at Peking University and Prof. Yi Zhang at Shanghai University. This work is supported by the National Key Research and Development Program of China, the National Natural Science Foundation of China, Collaborative Innovation Center of Quantum Matter, the Beijing Natural Science Foundation, the Innovation Program for Quantum Science and Technology, the Strategic Priority Research Program of Chinese Academy of Sciences, the China Postdoctoral Science Foundation and the U.S. Department of Energy, Basic Energy Sciences.
Source: School of Physics